Key Factors Influencing the Transfection Efficiency of Lipid Nanoparticle Formulations
- perryt6
- Feb 25
- 9 min read
Updated: Apr 8
Lipid nanoparticles (LNPs) have emerged as revolutionary delivery systems for nucleic acid therapeutics, most notably demonstrated by their critical role in mRNA COVID-19 vaccines. The transfection efficiency of these complex delivery vehicles depends on a multitude of interconnected factors, from their compositional architecture to cellular interactions. This report provides a comprehensive analysis of the key determinants that influence LNP transfection efficiency. It examines the intricate relationships between lipid components, physicochemical properties, formulation parameters, and biological interactions that collectively determine successful nucleic acid delivery to target cells.
Lipid Components and Their Optimal Ratios
The foundational architecture of lipid nanoparticles consists of a precise combination of four essential components, each contributing uniquely to the overall transfection capability. Understanding the role and optimal proportion of each component provides critical insights into designing high-efficiency LNP formulations.
1.1. Ionizable Cationic Lipids
Ionizable cationic lipids (Fig.1) represent the core functional component of modern LNP formulations, facilitating both nucleic acid encapsulation and endosomal escape[1]. These lipids possess a distinctive characteristic in their acid dissociation constants (pKa), which determines their ionization state and surface charge under different pH conditions.
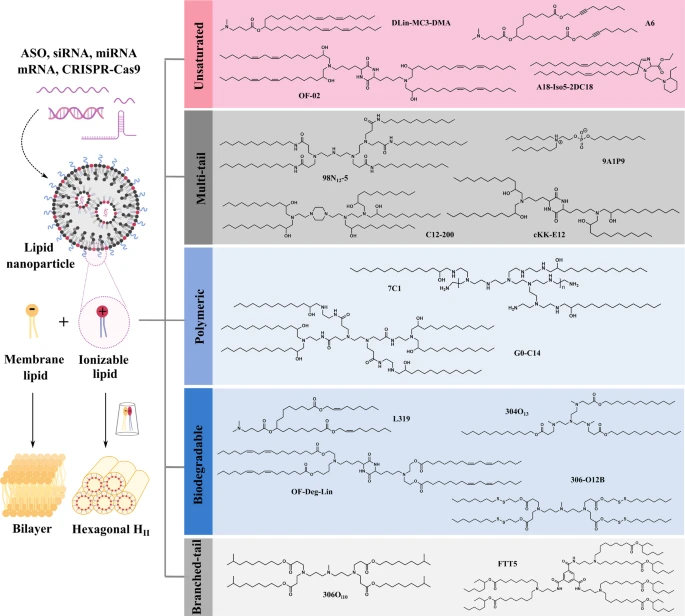
The landmark advancement from conventional permanently charged cationic lipids (such as DOTAP) to ionizable lipids has dramatically reduced toxicity concerns while improving circulation times. This improvement stems from reduced interactions with negatively charged serum proteins and decreased aggregation in the bloodstream[2]. Additionally, innovative lipid development has expanded beyond traditional structures, with compounds like iso-A11B5C1 demonstrating exceptional transfection efficiency comparable to established formulations while enabling more precise tissue targeting[3].
1.2. Helper Lipids and Phospholipids
Helper lipids and phospholipids play crucial roles in determining LNP membrane stability, fusion properties, and transfection efficiency[4]. Multiple studies have highlighted the significant impact of phospholipid selection on transfection outcomes across different cell types. Notably, DOPE (1,2-dioleoyl-sn-glycero-3-phosphoethanolamine) has emerged as a particularly effective helper lipid, functioning as a fusogenic component that promotes endosomal escape through membrane destabilization[5][6].

The chemical nature of these helper lipids substantially influences cell-specific transfection capabilities. Research has revealed that the choice between zwitterionic and cationic helper lipids can fundamentally alter the cellular uptake profile and subsequent transfection efficiency in specific cell populations[7]. This effect manifests differently across cell lines - one study demonstrated that phospholipid selection significantly affected transfection in MDA-MB-231 and SW 620 cancer cells but showed minimal impact on HEK 293T cells[6]. This differential response underscores the potential for helper lipid customization to achieve targeted delivery to specific cell populations.
1.3. Cholesterol
Cholesterol serves as a critical structural component in LNP formulations, enhancing membrane rigidity, reducing permeability, and improving overall particle stability.
Recent innovations have explored alternative formulations, including solid hydrophobic cores based on cholesterol acetate and coconut oil triglycerides, which demonstrated enhanced efficiency compared to squalene-based alternatives, though still less effective than conventional bilayer LNPs[6]. These findings highlight the nuanced role cholesterol plays in determining not just structural features but also functional transfection capabilities.
1.4. PEGylated Lipids
PEGylated lipids constitute a critical surface component that influences circulation time, colloidal stability, and immune recognition of LNPs. These lipids create a hydrophilic shield that prevents aggregation and reduces opsonization, thereby extending circulatory half-life[2][8]. The length of the PEG chain and the nature of the lipid anchor also significantly influence the dynamic properties of the LNP surface.
1.5. Optimal Component Ratios
The relative proportions of these four primary components profoundly impact transfection efficiency, though optimal formulations tend to display certain trends. The optimal molar ratio of cholesterol ranges from 20-40%, while PEGylated lipid is generally around 1-2%, ionizable lipid from 40-60%, and helper lipid the remainder.
Physicochemical Properties Affecting Transfection Efficacy
Beyond composition, the physical and chemical characteristics of LNPs strongly determine their transfection capabilities through influences on stability, cellular interactions, and intracellular processing.
2.1. Size and Polydispersity
Particle size and size distribution (polydispersity index, PDI) represent fundamental parameters affecting LNP tissue distribution, cellular uptake mechanisms, and transfection efficiency. Successful LNP formulations typically exhibit mean diameters between 70-200 nm with narrow size distributions (PDI < 0.2), allowing for effective endocytosis while avoiding rapid renal clearance.
2.2. Surface Charge and zeta Potential
Surface charge properties, often quantified as zeta potential, significantly impact LNP stability, protein interactions, and cellular uptake. Ionizable lipid-based LNPs exhibit pH-dependent charge transitions, remaining neutral at physiological pH (7.4) and positive charges in the acidic endosomal environment. Studies have shown that LNPs with zeta potentials around +30 to +40 mV often demonstrate optimal transfection properties.
Nucleic Acid Loading Efficiency
The loading efficiency and distribution of nucleic acids within LNPs significantly influence transfection outcomes. Recent research has revealed that LNPs exhibit heterogeneous nucleic acid content, with varying copy numbers of mRNA encapsulated per particle. Interestingly, fractionation studies demonstrated that the LNP fraction containing the highest mRNA loading levels actually exhibited the lowest transfection competence[9]. This counterintuitive finding suggests that optimal loading represents a balance between sufficient cargo delivery and maintaining the functional integrity of the LNP structure.
Formulation Parameters and Processing Conditions
The methods and conditions employed during LNP preparation significantly impact their final characteristics and transfection capabilities.
4.1 Buffer Composition and pH Considerations
Buffer composition during LNP formulation exerts substantial influence on nucleic acid packaging, particle stability, and ultimately transfection efficiency. The ionic environment during particle formation affects both the electrostatic interactions governing nucleic acid encapsulation and the structural organization of the lipid components. Research has identified that specialized buffer formulations, such as sodium potassium magnesium calcium and glucose solution (SPMCG), can significantly enhance mRNA transfer efficiency compared to conventional buffers[5].
4.2. Manufacturing Methods and Particle Formation
The method of LNP preparation fundamentally influences particle characteristics and transfection efficiency (Fig.3). Most clinically relevant LNP formulations employ rapid mixing techniques, where an ethanol solution containing lipids is rapidly combined with an aqueous solution containing nucleic acids under controlled conditions. This process allows for spontaneous particle assembly through a phenomenon described as fusion-dependent formation[10].

Microfluidic mixing has emerged as a revolutionary approach for LNP manufacturing, offering unprecedented control over particle formation processes and resulting characteristics. At its core, microfluidic mixing employs microscale chips with channels and chambers on the micrometer length-scales, where fluids are directed, mixed, and processed within a controlled environment. The fundamental principle involves the precise manipulation of fluid dynamics at microscale dimensions, where continuous laminar flow typically relies on diffusion between adjoining streams for mixing[11]. This controlled environment stands in stark contrast to turbulent mixing techniques, providing distinct advantages for research, development, and clinical manufacturing of LNP formulations.
Biological Barriers and Cellular Interactions
The journey from administration to successful transfection involves navigating multiple biological barriers, with cellular uptake mechanisms and endosomal escape representing particularly critical challenges.
5.1. Cellular Uptake Mechanisms
The mechanisms by which cells internalize LNPs fundamentally influence subsequent intracellular processing and transfection outcomes (Fig.4). Research has identified clathrin/dynamin-dependent endocytosis as a primary uptake pathway for many LNP formulations, with efficient formulations demonstrating enhanced engagement with this internalization route[9]. Previously discussed parameters such as particle size, surface properties, and lipid composition collectively determine the predominant uptake mechanism, allowing for potential optimization based on target cell characteristics.
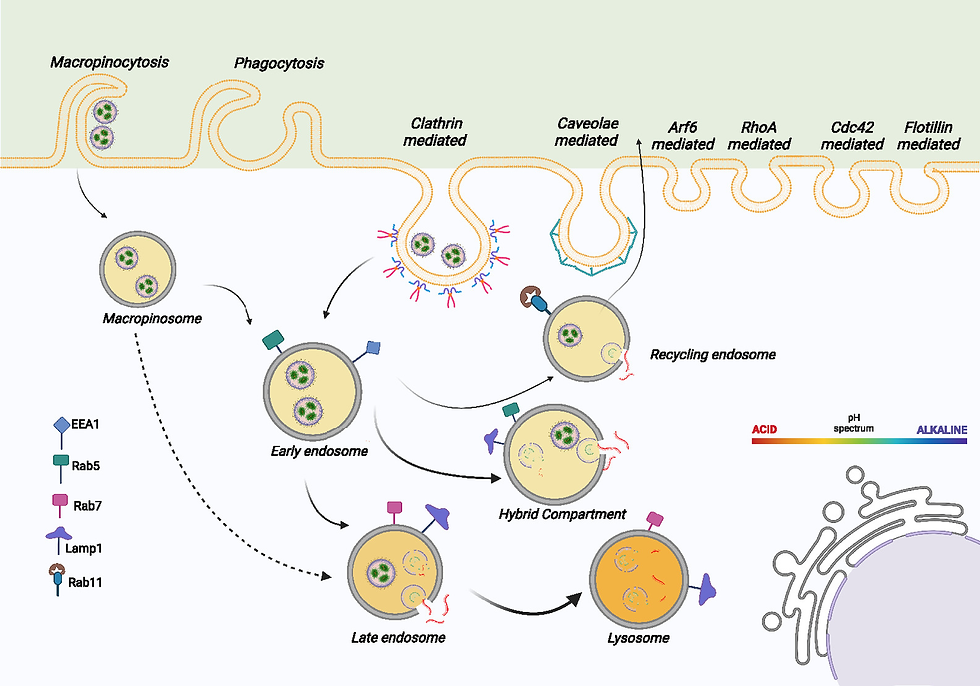
5.2. Endosomal Escape
Endosomal escape represents perhaps the most significant bottleneck in LNP-mediated transfection, highlighting the critical importance of enhancing endosomal release to improve overall transfection efficiency (Fig.5).
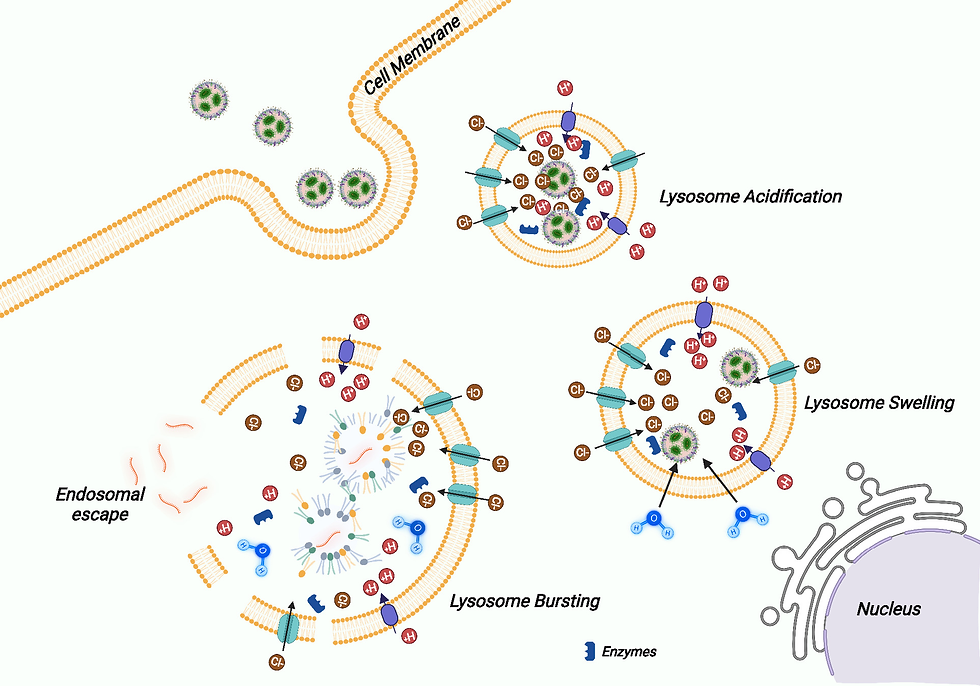
The ionizable lipid component plays a central role in facilitating endosomal escape through pH-dependent membrane disruption. As ionizable lipids become protonated, they promote interactions with the endosomal membrane that ultimately lead to disruption and cargo release. Strategies to enhance this process include incorporating lipids with optimized pKa values and structural features that promote membrane destabilization under acidic conditions.
5.3. Cell Type-Specific Considerations
Different cell types exhibit varying levels of susceptibility to LNP-mediated transfection, necessitating customized formulation approaches for specific applications. Factors including ionizable and helper lipid total molar percentage, N/P ratio, cholesterol to PEGylated lipid ratio, and helper lipid chemical identity have all demonstrated influence on cell type-preferential transfection[7]. These findings suggest potential strategies for developing targeted LNP formulations by manipulating compositional parameters rather than relying solely on surface ligand-based targeting (Fig.6).
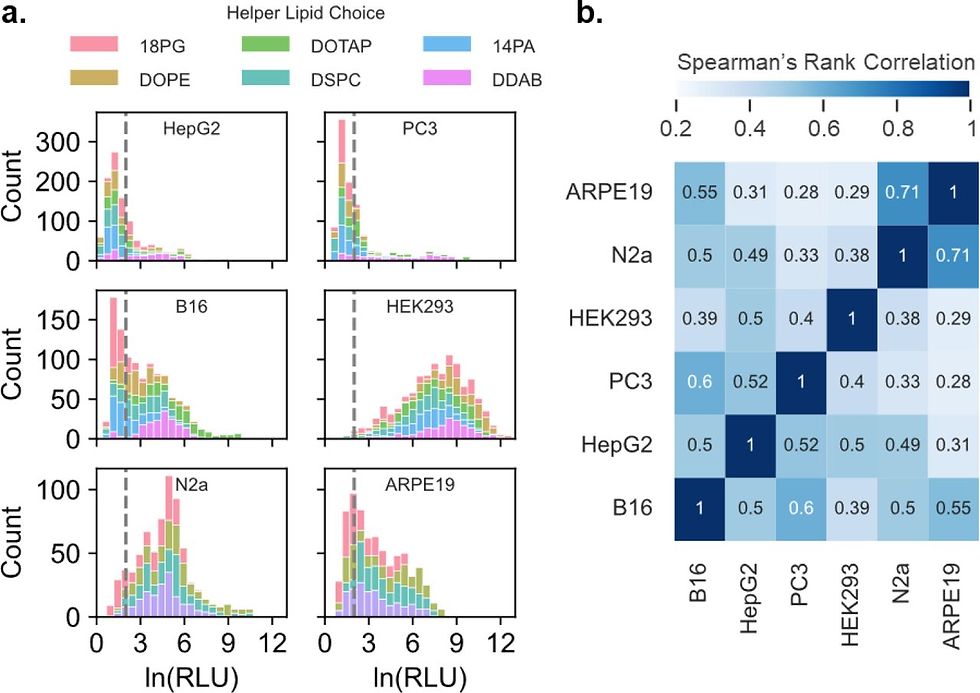
Research has demonstrated that the impact of phospholipid selection on transfection efficiency varies significantly across cell lines - affecting cancer cell lines like MDA-MB-231 and SW 620 but showing minimal influence in HEK 293T cells[6]. This differential response likely reflects variations in membrane composition, endocytic machinery, and intracellular trafficking pathways across cell types.
Advanced Optimization Approaches
The complexity of factors influencing LNP transfection efficiency necessitates sophisticated optimization strategies that can efficiently navigate the vast design space to identify optimal formulations.
6.1. High-Throughput Screening Platforms
Multi-step screening platforms have emerged as powerful approaches for efficiently identifying optimized LNP formulations from extensive candidate libraries. These platforms systematically address different physiological barriers in a stepwise manner, allowing for rapid down-selection of effective candidates from libraries containing thousands of potential formulations[12] (Fig.7). By incorporating sequential screening stages that evaluate different aspects of performance, these approaches can identify formulations with optimal characteristics for specific delivery applications.
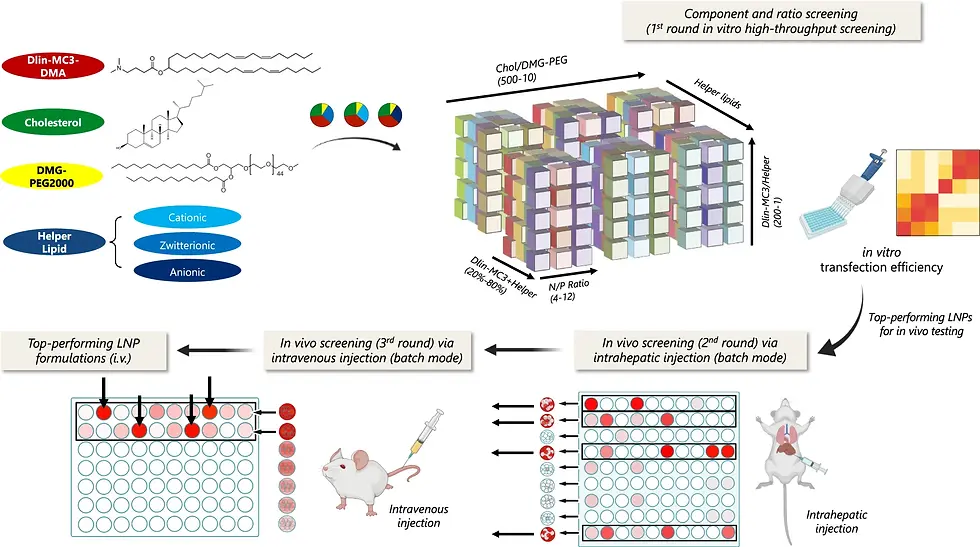
One example of successful implementation involved a screening platform that analyzed the role of different helper lipids and component ratios in plasmid DNA LNP-mediated gene delivery, successfully identifying formulations that achieved prolonged transgene expression in the liver following intravenous administration[12]. This systematic approach to formulation optimization represents a significant advancement over traditional trial-and-error methods.
6.2. Co-delivery Strategies for Enhanced Performance
Innovative co-delivery approaches combining nucleic acids with functional modulators have demonstrated significant potential for enhancing LNP performance. One notable example involved the co-delivery of plasmid DNA with siRNA targeting transcription factors regulating inflammatory response pathways, which substantially extended the duration of plasmid DNA nanoparticle-mediated transgene expression[12]. This approach highlights the potential for sophisticated cargo combinations to overcome biological limitations and enhance overall transfection outcomes.
Similarly, the co-encapsulation of small molecules that promote endosomal escape with nucleic acid cargo has shown promise for improving transfection efficiency. For instance, incorporating leukotriene inhibitors into mRNA-LNP formulations demonstrated significantly enhanced transfection both in vitro and in vivo[13]. These co-delivery strategies represent an important frontier in LNP development, potentially enabling improved performance through synergistic combinations of therapeutic and functional components.
6.3. Machine Learning Applications in LNP Design
Machine learning approaches have recently demonstrated tremendous potential for elucidating complex relationships between LNP composition and transfection performance. By analyzing data from high-throughput screening of compositionally diverse LNP libraries, machine learning algorithms can identify non-obvious patterns and design rules that inform rational formulation development[7].
Conclusion
The transfection efficiency of lipid nanoparticle formulations emerges from a complex interplay of compositional, physicochemical, and biological factors. Optimizing these diverse elements requires integrated approaches that address multiple aspects of the delivery process, from initial formulation design through cellular processing. The four core components of LNPs - ionizable lipids, helper lipids, cholesterol, and PEGylated lipids - must be carefully balanced in terms of both chemical identity and proportional ratios to achieve optimal performance. Physicochemical properties including size, surface charge, pKa values, and nucleic acid loading significantly influence particle-cell interactions and subsequent transfection outcomes. Formulation parameters and manufacturing methods establish the foundation for LNP functionality, while biological barriers - particularly endosomal escape - represent critical challenges that must be addressed to maximize delivery efficiency.
Advanced optimization strategies incorporating high-throughput screening, design of experiments, machine learning, and innovative co-delivery approaches are rapidly advancing our ability to develop LNP formulations with enhanced performance characteristics. As the field continues to evolve, these sophisticated development approaches hold tremendous promise for creating increasingly effective nucleic acid delivery systems for diverse therapeutic applications. The ongoing refinement of our understanding regarding the key factors influencing LNP transfection efficiency will undoubtedly drive the development of next-generation formulations with improved potency, specificity, and safety profiles, ultimately expanding the therapeutic potential of nucleic acid-based medicines.
References
An ionizable lipid toolbox for RNA delivery | Nature Communications
Machine Learning Elucidates Design Features of Plasmid Deoxyribonucleic Acid Lipid Nanoparticles for Cell Type-Preferential Transfection | ACS Nano
.